The capacity for crops to resist environmental and human-induced stressors governs their capacity to thrive in the modern world. With a growing population, inducing crop resistance has become a viable solution to improving food security.
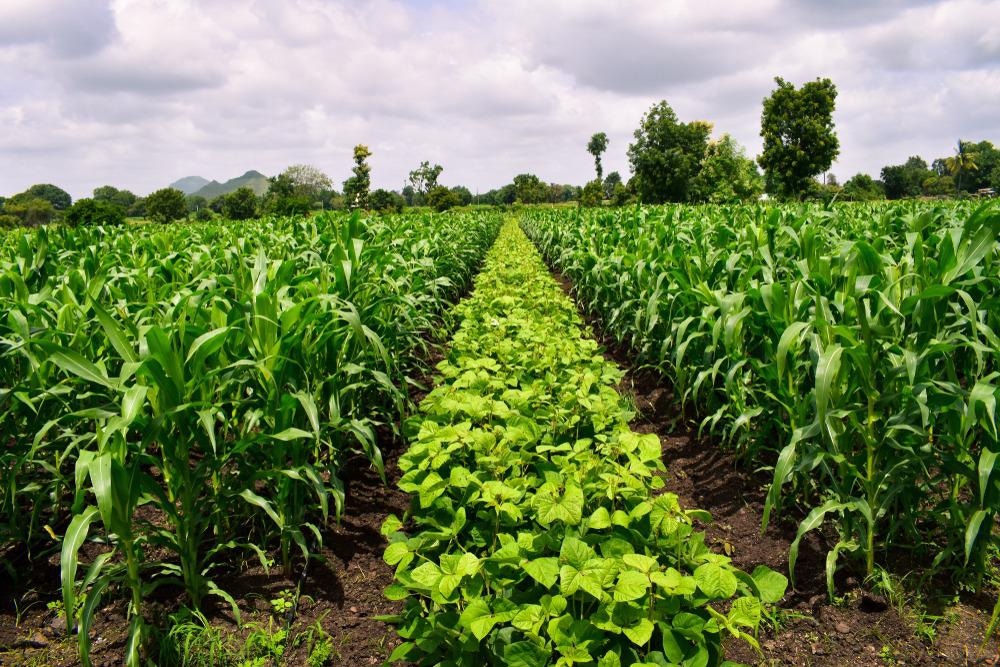
Image Credit: Alchemist from India/Shutterstock.com
The implications of crop resistance in an era of climate change and food insecurity
Crop resistance can be defined as the tolerance and resilience of plants to biotic and abiotic stress. Resistance can be affected by processes across biological levels of an organization, from gene regulation to individual metabolism and population-level turnover.
Such processes are essential for crop production as an estimated 26% of the worldwide crop production is lost each year pre-harvest due to the action of pests and pathogens, with further losses associated with climate and other stressors. Therefore, the improvement of crop resistance confers better chances of survival and recovery from biotic and abiotic stressors, which is essential in a world experiencing environmental changes combined with a growing need for food.
Traditionally, agricultural practices aimed to improve resistance by selecting heritable plant traits to reduce damage from pests, pathogens, droughts, and other similar instances. This was done through breeding techniques with the aim of increasing yield.
As our understanding of crop resistance improves, comparative studies of resistance are increasingly considered. Recent studies have found crop resistance is mostly a relative phenomenon, as genotypes can be more or less resistant than another genotype, which can be targeted to select for various factors.
In a special issue journal edition, Boscaiu and Fita, 2020 discuss how the study of plant responses to abiotic stress and stress tolerance mechanisms has become one of the most active research fields in plant biology. Mainly, the authors argue that abiotic stress is the primary stress for agriculture worldwide, making it a central factor affecting food production and human livelihood. Consequently, understanding the mechanisms conferring crop resistance to abiotic stress is central to ensuring the future of food security.
Gaining resistance through environmental drivers; soil microbiome
Tolerance and resilience to stress can be conferred to crops through biotic and abiotic factors, from evolutionary drivers such as genetic drift to thermal history and environmental factors. A 2020 study by Howard et al. considered how microorganisms in soil communities can provide stress-resistant crops.
Authors first discussed how soil microbiomes could promote plant growth by enhancing host tolerance to abiotic stresses, such as drought, and biotic stresses, such as pathogens and herbivores. Microorganisms are known to affect plants’ resistance to pathogens and herbivores through altering secondary metabolite production, and recent studies even show the rhizosphere microbiome may even serve as a stronger driver than plant genetics in determining plant resistance to insect herbivores. As a result, the soil microbiome is frequently proposed as a target for improving pest management in agriculture.
In this context, Howard et al. (2020) compared responses to microbiomes at various succession stages in 4 crops; maize (Zea mays, Poaceae), cucumber (Cucumis sativus, Cucurbitaceae), tomato (Solanum Lycopersicum, Solanaceae), and lettuce (Lactuca sativa, Asteraceae). Researchers assessed the growth and resistance of these crops with microbiome treatments to two generalist insect pests, cabbage looper (Trichoplusia ni) and fall armyworm (Spodoptera frugiperda).
The study found that microbiomes affected the growth and resistance of all plants to these pests, but the effects were species-specific. For example, the same microbiome that promoted leaf the most in lettuce also performed the worst in cucumber. Microbiome succession also affected crop resistance levels, with later succession microbiomes conferring the most resistance.
As a result, many agricultural practices are altering soil, plant, and insect microbial communities to favor crop resistance to produce healthier crops.
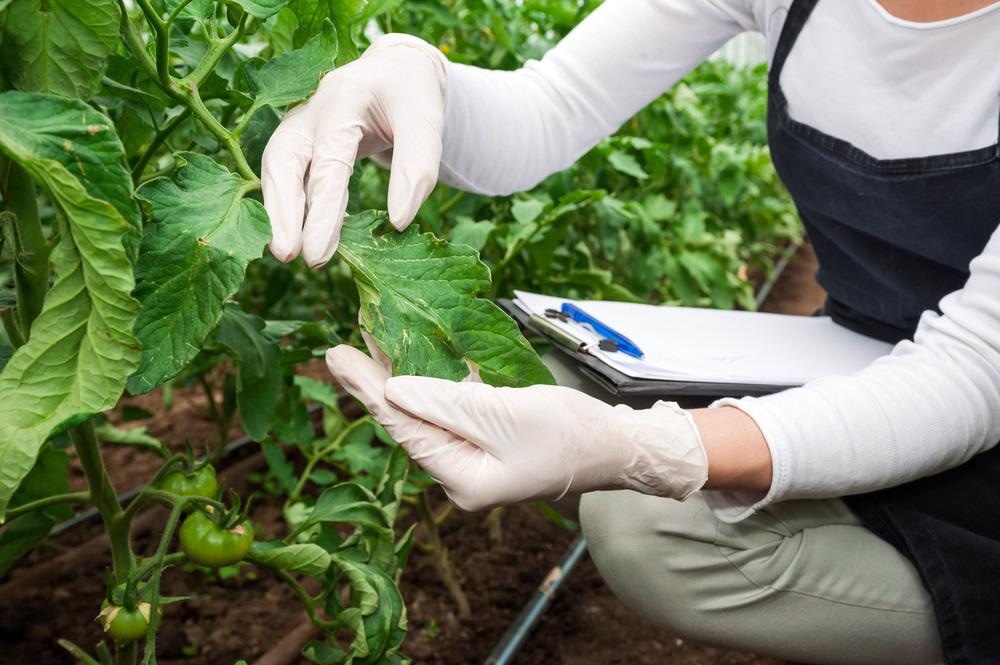
Image Credit: Vlad Teodor/Shutterstock.com
Human-induced crop resistance through gene editing
The advent of the golden age of molecular science has provided several pioneering technologies used to advance genetic modification in plants. Identifying, quantifying, and altering gene expression to favor traits enhancing resistance offers promising solutions to stabilizing food security, and such methods have occurred throughout agricultural practices over recent decades.
The history of crop resistance and resistance (R) genes to enhance resistance was reviewed by Piquerez et al., 2014. The study describes how traditional breeding has been selected for resistant crops for at least the last century, with the first mention of wheat disease resistance breeding programs dating from 1905. However, programs used resistance sources in crops by selecting for traits and crossing plants before understanding the mechanism of action of resistance genes.
Over the last 50 years, concepts of avirulence (Avr) gene processes in the pathogen and an R gene in the host plant emerged. The first Avr gene (AvrA) was eventually cloned, and the subsequent identification and cloning of single resistance loci of R genes ensued. Over time, the diverse number and type of methods used grew considerably.
A review of molecular techniques targeting crop resistance by Savadi et al., 2017, demonstrated how technologies are still improving and accelerating progress. For instance, in the last 20 years alone, methods such as marker-assisted selection and transgenic technology have provided highly new and effective strategies for enhancing resistance levels and durability in crop plants in a short period.
A number of existing biotechnological breeding methods have also improved considerably, including genomic selection, genome editing, cisgenesis and intragenesis, RNA-dependent DNA methylation, and reverse breeding. These techniques provide the ability to manipulate crop plants with greater precision and contribute to the acceleration of crop improvement efforts for sustained food production as well as overcoming safety concerns associated with food crops.
However, the authors mention how apprehensions of biosafety are gradually slowing the progress of transgenic applications in agriculture despite such progress. The public perception of genetic modifications in many countries remains uncertain, as ethics issues, alteration of natural gene pools, and health are still debated.
Nevertheless, genetic modification represents a key candidate for securing better food availability in a world experiencing changing environmental conditions, reduced soil quality, and a growing demand for food. The parallel application of other emerging technology alongside gene editing, such as computational modeling, will further provide beneficial tools at varying spatiotemporal scales to ensure food security.
Sources:
- Boscaiu, M., & Fita, A. (2020). Physiological and Molecular Characterization of Crop Resistance to Abiotic Stresses. Agronomy, 10(9), 1308. https://doi.org/10.3390/agronomy10091308
- Howard, M. M., Muñoz, C. A., Kao-Kniffin, J., & Kessler, A. (2020). Soil Microbiomes From Fallow Fields Have Species-Specific Effects on Crop Growth and Pest Resistance. Frontiers in Plant Science, 11. https://doi.org/10.3389/fpls.2020.01171
- Piquerez, S. J. M., Harvey, S. E., Beynon, J. L., & Ntoukakis, V. (2014). Improving crop disease resistance: lessons from research on Arabidopsis and tomato. Frontiers in Plant Science, 5. https://doi.org/10.3389/fpls.2014.00671
- Savadi, S., Prasad, P., Kashyap, P. L., & Bhardwaj, S. C. (2017). Molecular breeding technologies and strategies for rust resistance in wheat (Triticum aestivum) for sustained food security. Plant Pathology, 67(4), 771–791. https://doi.org/10.1111/ppa.12802
- Vyska, M., Cunniffe, N., & Gilligan, C. (2016). Trade-off between disease resistance and crop yield: a landscape-scale mathematical modelling perspective. Journal of The Royal Society Interface, 13(123), 20160451. https://doi.org/10.1098/rsif.2016.0451
Further Reading